Regeneration NYT article04/11/2006 10:04 AMRegrow Your Own - New York Times
April 11, 2006
Regrow Your Own
By NICHOLAS WADE
Stem cell therapy has long captured the limelight as a way to the goal of regenerative medicine, that of
repairing the body with its own natural systems. But a few scientists, working in a relatively obscure field,
believe another path to regenerative medicine may be as likely to succeed. The less illustrious approach is
promising, in their view, because it is the solution that nature itself has developed for repairing damaged
limbs or organs in a wide variety of animals.
Many species, notably amphibians and certain fish, can regenerate a wide variety of their body parts. The
salamander can regenerate its limbs, its tail, its upper and lower jaws, the lens and the retina of its eye,
and its intestine. The zebra fish will regrow fins, scales, spinal cord and part of its heart.
Mammals, too, can renew damaged parts of their body. All can regenerate the liver. Deer regrow their
antlers, some at the rate of 2 centimeters a day, said to be the fastest rate of organ growth in animals. In
many of these cases, regeneration begins when the mature cells at the site of a wound start to revert to an
immature state. The clump of immature cells, known as a blastema, then regrows the missing part,
perhaps by tapping into the embryogenesis program that first formed the animal.
Initiation of a blastema and the formation of the embryo are obviously separate biological programs, but
"the processes must converge at some point," says Jeremy Brockes, a leading regeneration researcher at
University College London.
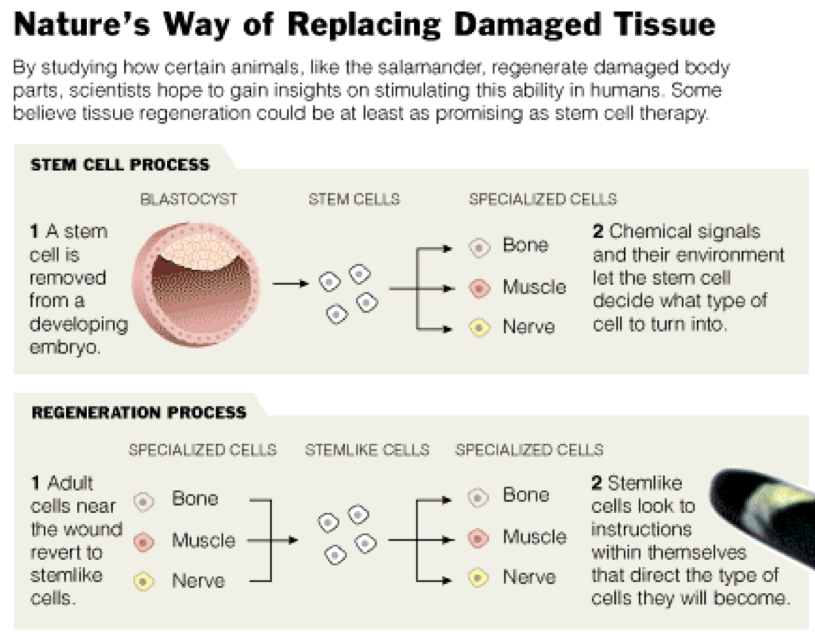
The blastema seems to derive its instructions from the wound-site cells from which it was formed, and is
quite impervious to cues from new surrounding tissue if it is transplanted. If a blastema made by
sectioning a salamander's limb at the wrist is transplanted elsewhere in the body it will still grow just a
wrist and paw, while a shoulder blastema will regrow the whole limb. People, of course, cannot regrow
their limbs like newts, and do not form blastemas, so the relevance of regeneration to medicine has long
seemed remote. But the capacity for regeneration exists in such a wide variety of species that it is unlikely
to have evolved independently in each, regeneration researchers believe.
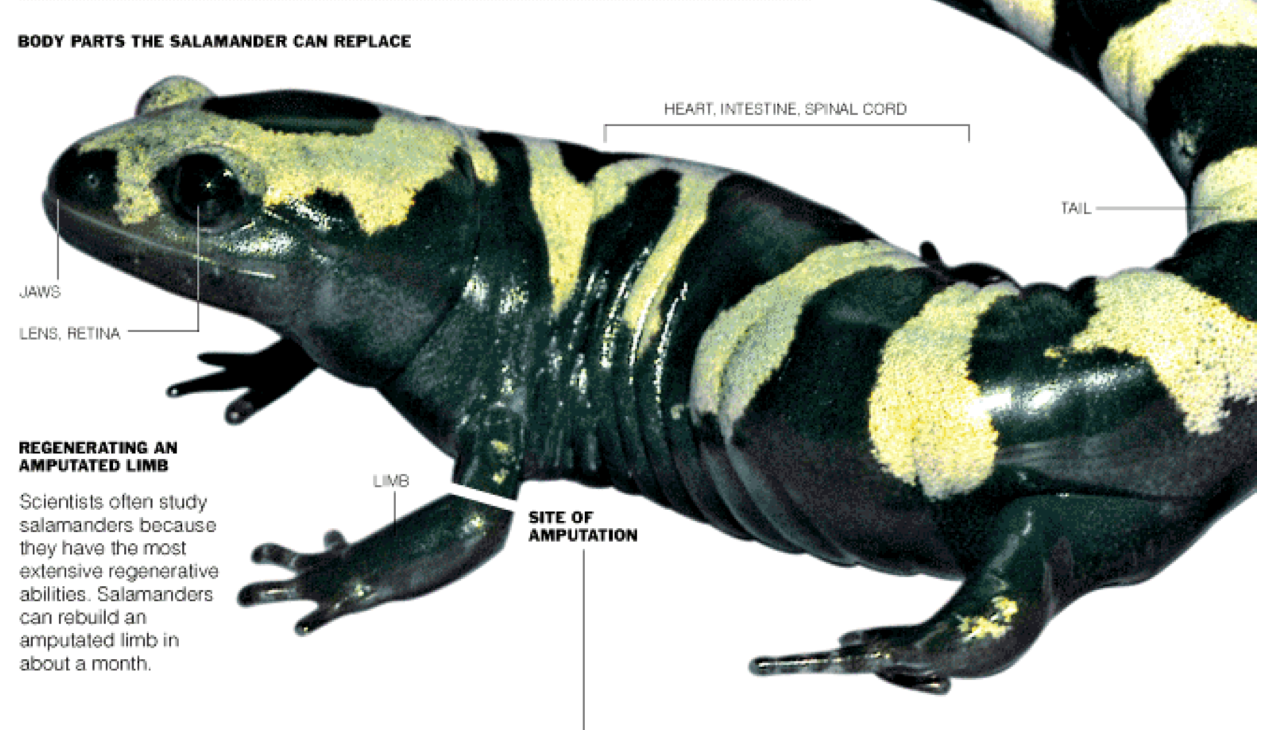
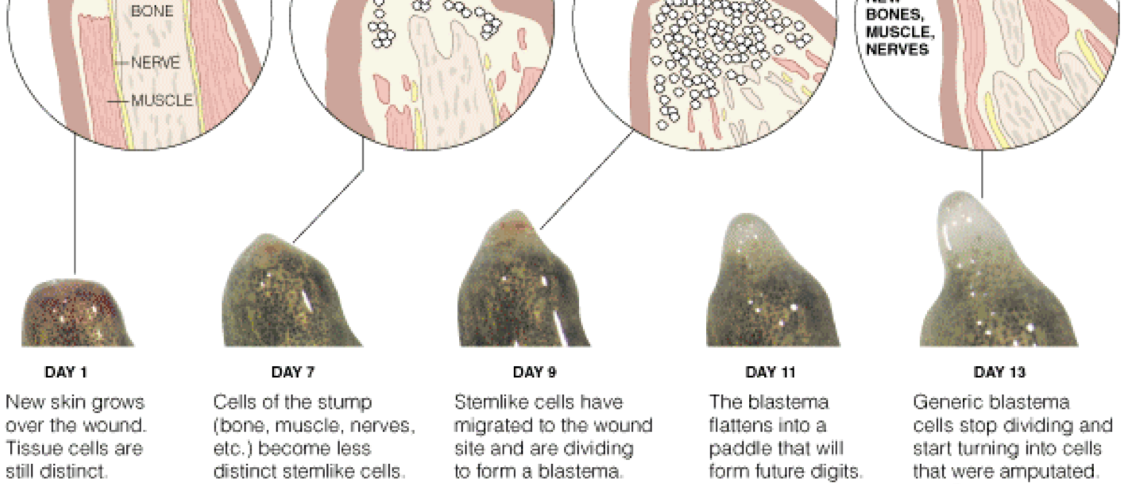 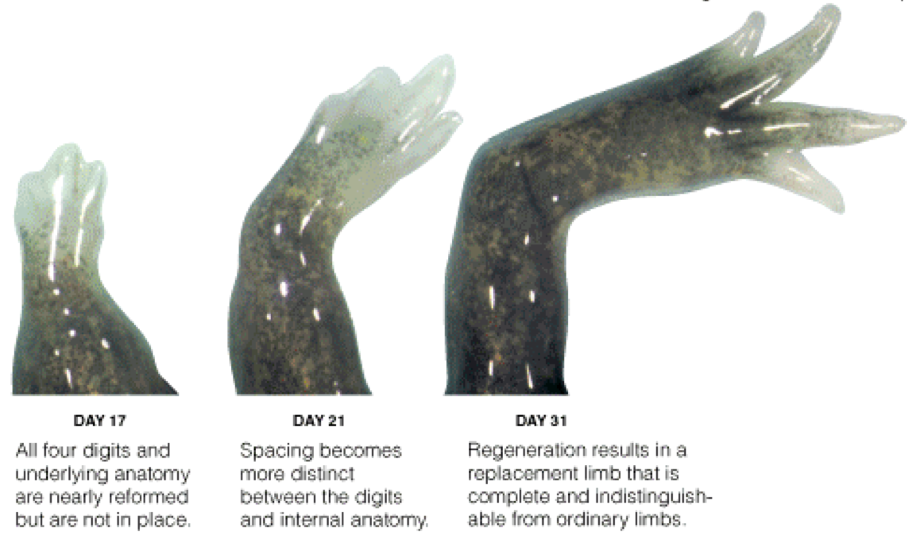
Rather, they say, the machinery regeneration must be a
basic part of animal genetic equipment, but the genes have for some reason fallen into disuse in many species.
In support of this notion, people are not wholly lacking in regenerative powers. There are reports
that the tip of the finger can occasionally be regenerated, if the cut is above the last joint. And people
can vigorously repair damage to the liver. Even after 75 percent has been removed in surgery, the liver
regains its original mass in two to three weeks. It is not certain why other organs and
limbs have lost this useful capacity, but perhaps only the liver was damaged often enough during its
owner's lifetime to make a repair system worth the cost. "I believe that the reason is the extensive and
recurring injury that the liver was exposed to in evolution: rotten food, plant toxins, viruses," says Markus
Grompe, a liver expert at the Oregon Health and Science University.
The liver can regenerate itself, when all else fails, from stem cells, the versatile cells that produce the
mature cells of many organs and tissues. But usually it relies on its own mature cells, which, like those of
a blastema, possess a remarkable power to divide and multiply, even though they can only restore the
organ's mass, not its original structure.
A more specific reason for thinking regeneration is not a wholly lost ability comes from genes. Last
December, Mark Keating, who studies regeneration in zebra fish, identified a gene that is essential for
initiating blastema formation when the fish's fin is cut. Both this gene, called fgf20, and another he has
found, hsp60, also exist in people, suggesting the genetic basis for regeneration may still be in place even
though the body can no longer evoke it.
Dr. Keating, a vice president at the Novartis Institutes for Biomedical Research in Cambridge, Mass.,
believes stem cells can ordinarily undertake only very limited repairs of organs like the liver and heart,
and that the scarring often seen in these tissues is a fallback mechanism put in place when the stem cells'
capacities are exceeded.
If the genes that boot up the zebra fish blastema also exist in people but are not switched on, perhaps
some drug might be developed that goads them into action. Once a blastema had been induced at some
wound site in the body, regeneration researchers suggest, it might regrow the missing limb or organ with
no further intervention required. "Maybe there are residual abilities that could be enhanced" in mammals,
says Shannon Odelberg, a researcher at the University of Utah. He studies regeneration in the newt, with
the eventual goal of inducing blastemas to form in mammals.
Regeneration is studied in only a few laboratories. It was not even on the agenda of the research planning
meeting held last October by the California Institute of Regenerative Medicine, which was dominated by
stem cell biologists. One reason for this orphan status is that the model animals used by most biologists,
like the roundworm, the fruitfly and the mouse, happen to be ones that do not regenerate.
The genetics of regenerating animals, like the salamander, are largely unknown. Hence the process of
regeneration has received little attention from research biologists. But there is a group of vertebrates that
can regenerate very successfully, said Dr. Brockes. "It would be rather surprising if there weren't some
interesting and important lessons one could learn from them."
"Regeneration is the result of an evolutionary experiment that nature has already done for us," said
Alejandro Sánchez Alvarado, a Hughes Institute researcher who studies flatworm regeneration at the
University of Utah The blastema, he notes, performs the difficult task one not faced by the embryo of
integrating new and existing tissues.
Many proponents of regeneration, while conceding they have a great deal more to learn, believe stem cell
therapy too may not be as close to clinical use as its advocates sometimes suggest. Dr. Brockes noted that
the blastema's reliance on internal information contrasts with a principal assumption of stem cell therapy,
that stem cells inserted into a damaged tissue will use local cues to behave appropriately and integrate
into the surrounding tissue.
Stem cell therapists assume that injected cells can replace missing tissue with guidance from the invisible
template supplied by chemical signals from nearby cells. That is the solution a human engineer might
logically think of, Dr. Brockes said, but evolution has chosen a different one.
The basic biology of regeneration is not yet fully understood, but nor is that of stem cells. Indeed, it may
be premature to start thinking about how to use stem cells therapeutically, said Dr. Sánchez Alvarado.
"Translating a biological process you don't understand into technology is like trying to translate
hieroglyphs without a Rosetta Stone," he said.
Dr. Grompe, the expert on liver regeneration, said that getting stem cells to behave properly in a patient's
body "is a very, very difficult problem." With transplanted stem cells, the usual outcome is "nonfunctional
at best and cancerous at the worst because the local environment is not able to modulate the behavior," he
said. "I think that cell therapy of the nervous system will be extremely difficult because of that. So much
for stem cells curing Alzheimer's."
Dr. Keating believes that the expense of stem cell therapy, should it work, is a major consideration. "I
would never begin to guess that the whole stem cell approach has no chance of working," he said. But
even if it does, developing cells for every patient who needs them would be very expensive. Switching on
the regenerative process with drugs, should that prove possible, would be cheap by comparison, he said.
Scientists who work on stem cells reject the idea that the blastema mechanism is the only way to repair
the body's tissues. "I agree that blastema regeneration models might have something to tell us, but I
wouldn't give up on normal stem cell regeneration," said Irving Weissman, a leading expert on blood stem
cells at Stanford University. The stem cells involved in bone marrow transplants "can regenerate drastic
loss of tissue," he said.
Bone marrow transplantation is the big success story on which much of the hope for stem cell therapy is
based. But regeneration researchers believe the bone-marrow example may be misleading because blood
is not an organized tissue, and the marrow's blood-making stem cells are not required to do anything
much beyond their usual function.
In disagreement with this view, Dr. Weissman said that blood-making stem cells are highly versatile and
have the ability to home in on the marrow and set up shop in their proper niche there, and that neural
stem cells appear to have a similar degree of versatility. Human neural stem cells, when put into
embryonic mice, will migrate through the mouse's brain and add insulation to mouse neurons that lack it.
Robert Weinberg, a biologist at the Whitehead Institute in Cambridge, said therapeutic regeneration was
"decades away" because the cells of animals that regenerate are so different from those of people.
But there is great hope of taking embryonic stem cells, he said, and making them yield primitive adult
stem cells that still possess regenerative capability. He placed less confidence in using fully mature adult
stem cells, which may have lost the ability to build new tissue. "I think the notion of trying to extract adult
stem cells from adult tissues is possibly a fool's errand," he said.
In the light of new knowledge, some stem cell biologists are making more guarded predictions about the
imminence of stem cell therapy. Ron McKay, an expert on neural stem cells at the National Institutes of
Health, noted that stem cells inserted into the developing brain of a fetal animal "become incorporated in
an extraordinary way, as if local cues were controlling their behavior."
But in the adult brain, he said, nothing happens, suggesting that the concept of using stem cells to treat
Alzheimer's disease is illusory.
Stem cells head the hierarchy of cells with which nature organizes animal tissues, but so much remains to
be understood that it is hard to tell which aspect of their biology may hold therapeutic promise. "I think
the idea of cell therapy per se will not be that powerful a tool for most diseases," Dr. McKay said. "But
stem cell biology will be a hugely important tool."
Regeneration and stem cell therapy are promising aspects of regenerative medicine but both are still at the
research stage. "I'm very bullish on regenerative medicine," said Dr. Keating, alluding to both types. "I
think it's going to happen and it will be a revolution, but it will take time. It would be a mistake to oversell
it and promise too much too early."
Copyright 2006 The New York Times Company
|
|
|
The human nervous system is an extraordinarily complex structure, and the things that we value most in life, such as cognition and being able to sense and interact with the external world, depend upon its proper wiring. Neurons transmit information along their axons, elongated processes analogous to cables, which connect their cell bodies to target cells. The nervous system can be divided into two distinct divisions, the central nervous system (CNS), which comprises the brain and spinal cord, and the peripheral nervous system (PNS), which consists of the peripheral nerves that innervate the body. One of the ways in which these two compartments of the nervous system markedly differ lies in their capacity to regenerate after injury. In stark contrast to the PNS, where severed axons often will heal and successfully navigate back to their original targets, injured CNS neurons exhibit a burst of stymied growth but ultimately fail, with their axons stalling out and forming distinctive large endings dubbed ‘retraction bulbs’ that fail to transverse the site of injury (Figure 1). These morphological changes are thought to reflect regeneration failure. Although axotomy can cause neuronal death, many neurons will survive months to years after injury, particularly if their axons have been severed far from their cell bodies.
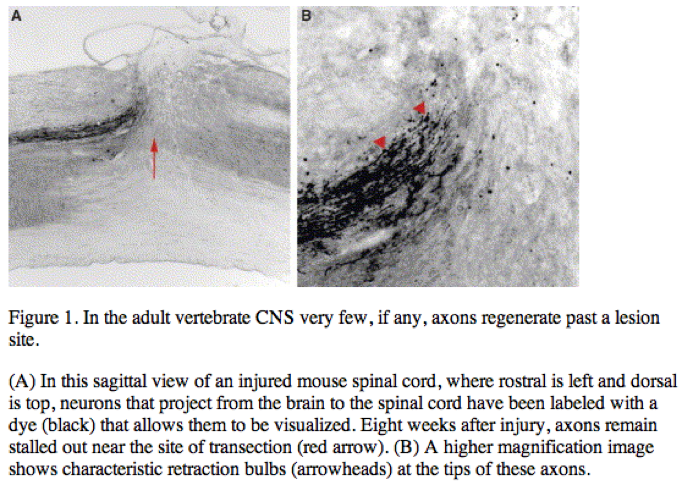
Clinically, the CNS’s inability to re-wire after injury is exemplified by patients who have suffered a spinal cord injury. Although damage to the spinal cord causes general trauma, killing neurons and supporting glial cells at the site of injury, this disorder can be viewed as primarily a re-wiring problem as most pathological symptoms, including numbness and paralysis, reflect the permanent interruption of the flow of information from ascending sensory and descending motor tracts. Injuries to the lower spinal segments generally render patients unable to walk or control their bladder and bowels, while injuries to the cervical levels of the spinal cord can cause additional deficits including the loss of arm movement and an inability to breathe without a respirator. In addition to obvious quality-of-life concerns, the CNS’s failure to rewire has enormous clinical ramifications for such patients, as reduced mobility often precipitates fatal complications including pneumonia, pulmonary emboli and septicemia.
Current therapies aim to save remaining intact fibers by minimizing secondary damage. The standard treatment often involves stabilization of the spine and administration of methylprednisolone, a steroidal drug that minimizes inflammation and may decrease the number of neurons that die as a result of swelling. After this initial pharmacological intervention, treatment is limited to physical therapy or, more rarely, experimental stem cell transplants. There is great optimism in the field that understanding more about the molecular mechanisms that prevent CNS regeneration will spur targeted therapies that will shift treatment goals from palliative care to regeneration and restoration of function after injury.
Reasons for optimism
Why do many scientists believe that, with appropriate intervention, CNS neurons would be capable of regeneration? One could easily imagine that central neurons are simply hard-wired in such a way that they will not repair under any circumstance. Several lines of evidence argue against this possibility. First, as touched upon earlier, axons in the PNS are capable of regenerating after injury. This is an interesting phenomenon, as many neurons with processes inside the PNS also have an axon or part of an axon within the CNS, yet only the peripheral portions are able to regenerate. These paradoxical growth responses suggest that the neurons’ interactions with the two different environments may contribute to their dissimilar regenerative responses and that the PNS, but not the CNS, environment is a permissive substrate for regenerative outgrowth.
Furthermore, numerous comparative studies have revealed phylogenetic differences in the capacity of different species to regenerate. Whereas axons in the CNS of warm-blooded vertebrates (mammals and birds) do not regenerate, those in many lower vertebrates, including newts and salamanders, can regenerate after injury. Very young mammals, birds and certain amphibians are also often capable of substantial CNS repair [1]. These data suggest that the lack of CNS regeneration in vertebrates is the result of a recent evolutionary change, although it is still unclear whether these varied responses are caused by differences in expression of genes that are conserved across these organisms or by the presence of proteins specific to warm-blooded vertebrates.
Although one hesitates to draw too many conclusions from these disparate data, observations of robust regrowth in peripheral nerves, and in the central tracts of young mammals and in adult lower vertebrates, lend hope that the constraints on regeneration involve sufficiently few players that pharmacological interventions might be designed that can stimulate injured tracts to regenerate functionally.
Anatomy of the injury site
CNS axons are normally surrounded by insulating layers of lipids called myelin sheaths, made by a type of cell called an oligodendrocyte. After injury, the severed tips of these neurons encounter a varied terrain as they attempt to regenerate, contacting components present in the intact CNS as well as those unique to the injured CNS. Following traumatic insult, the distal portion of a severed axon will degenerate and the resulting debris from it and its surrounding myelin sheath persists along the lengths of the degenerated tracts until the fragments are cleared by macrophages as well as another type of cell termed microglia.
Although they share many structural similarities, the PNS environment differs from that of the CNS. To begin with, PNS axons are myelinated by Schwann cells rather than oligodendrocytes, glial cells that are morphologically and physiologically distinct from their CNS myelinating counterparts. CNS and PNS injury can also be distinguished by how quickly degenerating tissue is eliminated. The cleaning-up of axon fragments and myelin debris is many times more efficient in the PNS than in the CNS, a feature probably explained by the involvement of different cellular players as well as differential access to the immune system.
The histology of a lesion varies somewhat depending on the type of injury sustained, but generally in the CNS the epicenter is quickly invaded by a host of cell types, including fibroblasts, vascular endothelial cells and macrophages. Surrounding this area is a zone of glial cells filled with astrocytes, oligodendrocyte precursors and microglia. Astrocytes in this region become markedly hypertrophic, and these ‘reactive astrocytes’ go on to form a dense network called a glial scar. This particular scarring response is unique to the CNS, as there are no astrocytes in the PNS.
In the field’s effort to define what goes wrong after injury, the neurons’ intrinsic growth state, the glial scar, myelin debris, and invading cells from the periphery have all in turn been investigated as likely suspects involved in inhibiting CNS regeneration.
Barriers to regeneration
Embryonic and adult neurons differ substantially in their intrinsic growth potential, with their axons switching perinatally from an elongating to a more branching phenotype. This shift in growth ability led researchers to postulate that the intrinsic growth state of adult CNS neurons disfavors regeneration [2]. Although mammalian central neurons generally do not regrow after injury, particular situations have been described which ‘precondition’ these cells for later regenerative outgrowth. This phenomenon has been documented in a type of bipolar sensory neurons, cells whose peripheral, but not central, branch normally regenerates after injury. However, if the peripheral branch is lesioned before the central branch, the neuron is somehow ‘primed’ such that the central fiber will now sprout some distance after a CNS lesion, showing that the central branch is indeed capable of regeneration [3 and 4]. The manipulation of signaling pathways by elevating the level of cyclic (c)AMP can similarly change a neuron’s propensity to regenerate. Indeed, CNS regeneration can be enhanced in vivo by delivering a cAMP analog or by administering rolipram, which inhibits an enzyme that blocks the breakdown of cAMP [5].
Keeping in mind that intrinsic factors play an important role, classic experiments by Aguayo and colleagues [6] argue that environmental cues also ultimately dictate whether or not an adult neuron will regenerate after injury. In a clever set of experiments these researchers used segments of PNS nerve to connect rats’ spinal cords with their brain stems. Remarkably, they observed that many CNS axons grew out from the spinal cord more than three centimeters into this peripheral nerve graft. This experiment was particularly exciting because it showed that, in a suitable environment, CNS neurons are capable of extending processes as adults. This recognition galvanized efforts to determine which molecular differences between the PNS and CNS environment makes the latter inhospitable for the re-growth of adult neurons. While the CNS may lack certain positive factors present in the PNS [7], much attention has focused on molecules present in the CNS that actively block regeneration.
The glial scar
Several lines of evidence suggest that the glial scar contributes to regeneration block [8]. When dissociated adult neurons were injected into rats that had sustained CNS injuries, researchers observed that many of these transplanted cells grew considerable distances, hindered only when they approach the glial scar. The addition of growth factors similarly increased the ability of CNS axons to grow, but they still stalled out at the scarred region. After traumatic insult, a number of extracellular molecules of the chondroitin sulfate proteoglycan (CSPG) family are elevated in the scar tissue, leading researchers to propose these molecules as candidates for mediating the inhibitory activity of the scar.
Consistent with this possibility, the application of chondroitinase ABC (chABC), an enzyme which selectively degrades CSPGs, leads to clear regeneration in vivo following various types of CNS lesion [8 and 9]. This negative effect of CSPGs on regenerative outgrowth may reflect a direct effect on the axons. Alternatively, as these highly charged molecules bind many molecules, it is conceivable that neurons are inhibited by distinct factors that associate with CSPGs. As chABC treatment has been shown to be a robust and reproducible method of increasing CNS regeneration, this line of research should prove exciting to follow in coming years as the molecular players are delineated.
Inhibitory proteins within CNS myelin
As previously mentioned, the clearance of myelin debris is extremely slow within the adult CNS. As these remnants persist for weeks and months after injury, the possibility was raised that residual myelin contains factors that actively prevent injured neurons from regenerating. Numerous in vitro experiments are consistent with this hypothesis; for example, dissociated neurons are impeded from extending axons when plated on either purified myelin extracts or substrates of myelin-rich CNS tissue [10 and 11]. Various approaches that target myelin lead to some regeneration in vivo; examples include experiments where animals were first irradiated in order to impair the formation of myelin-producing oligodendrocytes, or immunized with myelin extracts [11].
Over the past decade three prominent myelin-derived inhibitors have been identified: myelin associated glycoprotein (MAG), Nogo-A, and oligodendrocyte myelin glycoprotein (OMgp) [12]. Surprisingly, although these three inhibitors are structurally distinct, work from the last several years has suggested that all three bind a receptor complex containing the Nogo receptor (NgR), the low affinity neurotrophin receptor (p75NTR) and/or the p75NTR relative TROY [13]. Signaling through this receptor complex is thought to inhibit neurite outgrowth by activating RhoA and ROCK, molecules known to regulate cytoskeletal dynamics [14]. Hopes remain high that this striking convergence of inhibitory signaling pathways may allow for simultaneous targeting of multiple inhibitory constituents of CNS myelin.
Knockout mice for many of these molecules, including Nogo, MAG, NgR and p75NTR have been generated, and mutant animals have also been assessed for their potential to regenerate after injury. Although some lines demonstrate evidence of increased regeneration, the absence of a robust regenerative phenotype in others suggests that redundancies in myelin cues or synergy with other inhibitory factors ultimately prevent successful regrowth ([15 and 16] and references therein). In general, the relative contributions of each of these individual factors to regeneration block remains unclear. Future analysis of various double and triple knockout animals should be instructive.
Guidance molecules as contributors to regeneration block
Other factors that are strong candidates for contributing to regeneration block are members of the netrin, semaphorin, ephrin and slit families of axon guidance molecules. Many of these proteins instruct neurons’ directional choices during embryonic development through repulsive or inhibitory actions on axons [17]. Aspects of successful regeneration recapitulate developmental events, and the potent ability of some of these molecules to repulse embryonic axons underscores the possibility that they inhibit regeneration in the adult. Although many axon guidance molecules are downregulated when development is complete, some of them remain expressed in the adult, and others are reexpressed following injury in components of the glial scar, in myelin, or in gray matter [18 and 19].
The contributions of these molecules to regeneration block are only now starting to be defined. In one recent study [20], striking regeneration was observed following spinal cord injury in mice mutant for an ephrin receptor, EphA4. Various members of the semaphorin family of molecules are highly expressed after injury, and recent in vitro work has shown that one of these proteins, Sema5A, likely contributes to the regeneration block of neurons which project from the retina to the brain [18 and 21]. Many of these repulsive axon guidance ligands and their receptors are expressed in patterns consistent with their playing a role in the regeneration block; in particular, netrin-1, Sema4D and ephrinB3 have all been shown to be expressed by CNS myelin, and evidence has been obtained that Sema4D and ephrinB3 contribute to the inhibitory activity of myelin in vitro [19, 22 and 23]. It will be important to ascertain to what extent they contribute to the observed stalling out of axons after injury.
Conclusions
Despite the passage of forty-five centuries since an anonymous ancient Egyptian described the catastrophic effects of CNS injury [24] (Figure 2), it wasn’t until the last 20 years that we have begun to understand some of the molecular events that contribute to regeneration block. As researchers continue to define and characterize the critical barriers to regeneration, it will be interesting to assess to what extent recovery is possible. Many investigators have been pondering and started to tackle critical ‘next-step’ questions. Once past the lesion site, do regenerating fibers successfully navigate back to their former target cells? If so, do they form functional synapses? Are treatment strategies that repair a clean knife-swipe (cut) lesion pertinent to the complex contusion (crush) injuries that comprise the vast majority of human cases? Will therapies be limited to recent injuries, or might people benefit who have sustained spinal cord damage years or even decades before?
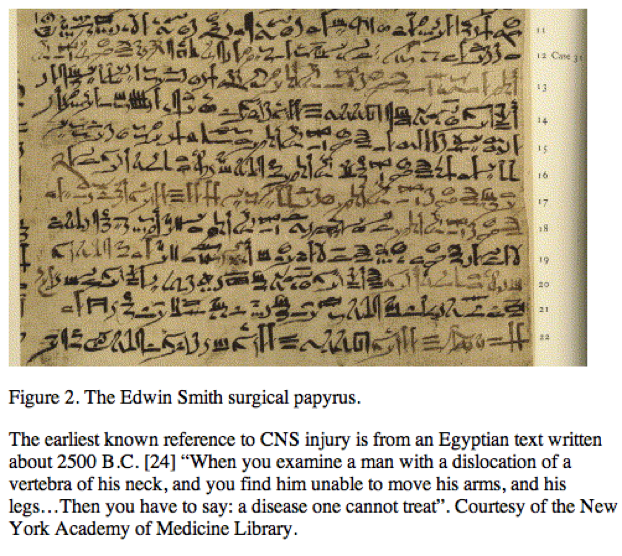
This is clearly a time of great excitement and hope and a belief that understanding the molecular processes that prevent regeneration could shift treatment options from palliative care to targeted restorative therapies. Although it may prove difficult to completely recover lost function, successfully encouraging neurons to grow just a spinal segment or two could translate into dramatic quality-of-life improvements. For instance, short-distance restoration of spinal circuitry could allow patients with cervical injuries to breathe independently without a respirator, or those who have sustained lumbar injuries to increase mobility and regain bowel and bladder function. The field of CNS regeneration is alive and bursting with potential; the next decade holds the promise of exciting progress.
References
1 P. Ferretti, F. Zhang and P. O’Neill, Changes in spinal cord regenerative ability through phylogenesis and development: lessons to be learnt, Dev. Dyn. 226 (2003), pp. 245256. Full Text via CrossRef
2 J.L. Goldberg, M.P. Klassen, Y. Hua and B.A. Barres, Amacrine-signaled loss of intrinsic axon growth ability by retinal ganglion cells, Science 296 (2002), pp. 18601864. Full Text via CrossRef
3 S. Neumann and C.J. Woolf, Regeneration of dorsal column fibers into and beyond the lesion site following adult spinal cord injury, Neuron 23 (1999), pp. 8391. SummaryPlus | Full Text + Links | PDF (629 K)
4 T. Spencer and M.T. Filbin, A role for cAMP in regeneration of the adult mammalian CNS, J. Anat. 204 (2004), pp. 4955. Full Text via CrossRef
5 L.M. Ramer, M.S. Ramer and J.D. Steeves, Setting the stage for functional repair of spinal cord injuries: a cast of thousands, Spinal Cord 43 (2005), pp. 134161. Full Text via CrossRef
6 S. David and A.J. Aguayo, Axonal elongation into peripheral nervous system “bridges” after central nervous system injury in adult rats, Science 214 (1981), pp. 931933.
7 M.S. Ramer, J.V. Priestley and S.B. McMahon, Functional regeneration of sensory axons into the adult spinal cord, Nature 403 (2000), pp. 312316. Full Text via CrossRef
8 J. Silver and J.H. Miller, Regeneration beyond the glial scar, Nat. Rev. Neurosci. 5 (2004), pp. 146156. Full Text via CrossRef
9 E.J. Bradbury, L.D. Moon, R.J. Popat, V.R. King, G.S. Bennett, P.N. Patel, J.W. Fawcett and S.B. McMahon, Chondroitinase ABC promotes functional recovery after spinal cord injury, Nature 416 (2002), pp. 636640. Full Text via CrossRef
10 P. Caroni and M.E. Schwab, Two membrane protein fractions from rat central myelin with inhibitory properties for neurite growth and fibroblast spreading, J. Cell Biol. 106 (1988), pp. 12811288. Full Text via CrossRef
11 Z. He and V. Koprivica, The Nogo signaling pathway for regeneration block, Annu. Rev. Neurosci. 27 (2004), pp. 341368. Full Text via CrossRef
12 M.T. Filbin, Myelin-associated inhibitors of axonal regeneration in the adult mammalian CNS, Nat. Rev. Neurosci. 4 (2003), pp. 703713. Full Text via CrossRef
13 W.J. Mandemakers and B.A. Barres, Axon regeneration: it’s getting crowded at the gates of TROY, Curr. Biol. 15 (2005), pp. R302R305. SummaryPlus | Full Text + Links | PDF (107 K)
14 D.H. Lee, S.M. Strittmatter and D.W. Sah, Targeting the Nogo receptor to treat central nervous system injuries, Nat. Rev. Drug Discov. 2 (2003), pp. 872878.
15 C.J. Woolf, No Nogo: now where to go?, Neuron 38 (2003), pp. 153156. SummaryPlus | Full Text + Links | PDF (44 K)
16 B. Zheng, J. Atwal, C. Ho, L. Case, X.L. He, K.C. Garcia, O. Steward and M. Tessier-Lavigne, Genetic deletion of the Nogo receptor does not reduce neurite inhibition in vitro or promote corticospinal tract regeneration in vivo, Proc. Natl. Acad. Sci. USA 102 (2005), pp. 12051210. Full Text via CrossRef
17 M. Tessier-Lavigne and C.S. Goodman, The molecular biology of axon guidance, Science 274 (1996), pp. 11231133. Full Text via CrossRef
18 F. De Winter, M. Oudega, A.J. Lankhorst, F.P. Hamers, B. Blits, M.J. Ruitenberg, R.J. Pasterkamp, W.H. Gispen and J. Verhaagen, Injury-induced class 3 semaphorin expression in the rat spinal cord, Exp. Neurol. 175 (2002), pp. 6175. Abstract | Abstract + References | PDF (1054 K)
19 P.D. Koeberle and M. Bahr, Growth and guidance cues for regenerating axons: where have they gone?, J. Neurobiol. 59 (2004), pp. 162180. Full Text via CrossRef
20 Y. Goldshmit, M.P. Galea, G. Wise, P.F. Bartlett and A.M. Turnley, Axonal regeneration and lack of astrocytic gliosis in EphA4-deficient mice, J. Neurosci. 24 (2004), pp. 1006410073. Full Text via CrossRef
21 J.L. Goldberg, M.E. Vargas, J.T. Wang, W. Mandemakers, S.F. Oster, D.W. Sretavan and B.A. Barres, An oligodendrocyte lineage-specific semaphorin, Sema5A, inhibits axon growth by retinal ganglion cells, J. Neurosci. 24 (2004), pp. 49894999. Full Text via CrossRef
22 C. Moreau-Fauvarque, A. Kumanogoh, E. Camand, C. Jaillard, G. Barbin, I. Boquet, C. Love, E.Y. Jones, H. Kikutani and C. Lubetzki et al., The transmembrane semaphorin Sema4D/CD100, an inhibitor of axonal growth, is expressed on oligodendrocytes and upregulated after CNS lesion, J. Neurosci. 23 (2003), pp. 92299239.
23 M.D. Benson, M.I. Romero, M.E. Lush, Q.R. Lu, M. Henkemeyer and L.F. Parada, Ephrin-B3 is a myelin-based inhibitor of neurite outgrowth, Proc. Natl. Acad. Sci. USA 102 (2005), pp. 1069410699. Full Text via CrossRef
24 J.H. Breasted, The Edwin Smith surgical papyrus, University of Chicago Press, Chicago (1980).
Current Biology: Volume 15, Issue 18 , 20 September 2005, Pages R749-R753
|
|